How to make the most of carbon dioxide
On 29 September, the XPRIZE Foundation based in Culver City, California, announced a 4½-year competition that will award US$20 million to the research team that can come up with the best way to turn carbon dioxide from a liability into an asset.
With gigatonnes of the gas pouring into the atmosphere each year, and with the consequences for global climate becoming increasingly obvious, the Carbon XPRIZE would reward technologies that can convert CO2 emissions from coal and natural-gas power plants into useful products such as alternative building materials, fuels and raw material for the manufacture of plastics and other chemicals.
The invitation should have plenty of takers: a growing number of companies and research chemists are already pursuing that goal. In Reykjavik, what looks like an overgrown playground climbing frame is actually a small chemical plant that turns CO2 into methanol: a fuel that can also be used to manufacture products ranging from paints to wrinkle-resistant textiles. In Houston, Texas, another small plant is turning CO2 into materials that go on to become coatings and adhesives. And in Tokyo, Japan, Asahi Kasei Chemicals is widely licensing its technique for turning CO2 into the polycarbonate plastics used in bulletproof glass, spectacle lenses and electronic parts.
Using this greenhouse gas as a raw material is an idea that many scientists once dismissed as hopeless, says Chunshan Song, a chemical engineer at Pennsylvania State University, University Park. As a practical matter, he says, “lots of people believed that nothing could be done with CO2 utilization” after the stuff went up the smokestack.
As a source of carbon, sceptics argued, the gas was far more difficult and expensive to obtain than the petroleum, coal and natural gas that now provide the raw material for most chemical manufacturing. And even if CO2 could be captured cheaply enough, converting such a stable molecule into more-useful chemicals would generally require lots of energy, which might well come from fossil-fuel plants. The conversion could cost a fortune and make more CO2 than it consumed.
But the balance is starting to shift. New conversion technologies are allowing the energy-hungry chemical reactions to proceed more efficiently. Renewable sources such as solar and wind can increasingly supply that energy at a competitive cost without the carbon penalty. And solutions to the capture problem may be forthcoming if governments follow the recommendations of the Intergovernmental Panel on Climate Change (IPCC) and mandate carbon capture and storage — grabbing the CO2 coming out of power plants and other industries, and locking it away underground. But rather than simply burying it, companies could defray the cost of capture by putting the gas to productive use.
Michele Aresta, a chemist at the University of Bari in Italy, estimates that if currently known processes were deployed most efficiently and at the greatest possible scale, they could directly use some 300 million tonnes of CO2 per year, while indirectly reducing emissions by around a gigatonne per year — roughly 5% of the total net emissions1. This is hardly a total solution to the CO2 problem, he says, but it is substantial nonetheless. And that is not counting the additional benefits, which include a shift away from relying on fossil fuels as a source of carbon for materials and chemicals, as well as the use of CO2-derived fuels to store and transport energy from wind, solar and other intermittent sources.
The Carbon XPRIZE may well accelerate these developments. But research into CO2 conversion has already been receiving support from funding agencies worldwide. Since 2009, for example, the German Ministry of Education and Research has pumped in around €100 million (US$110 million) to support research on the use of CO2 in chemical and synthetic-fuel production. In 2011, the US Department of Energy invested $106 million in various CO2-utilization projects. The European Union has lined up a 2020 Horizon Prize worth €1.5 million for a technology that demonstrates viable CO2 reuse. And over the next five years, China is expected to invest some 30 billion yuan ($4.7 billion) in CO2 recycling in the coal, steel, cement and paper industries.
Low-hanging fruit
Industrial uses of CO2 have been around for generations. Every year, about 20 million tonnes of the gas are used ‘as is’: for example, it’s the ‘fizz’ in fizzy drinks, and it can also be used as a high-pressure solvent to clean electronics. But gas used in this way quickly returns to the atmosphere, where it contributes to global warming.
Another 114 million tonnes of CO2 go into the production of urea fertilizer every year, accounting for more than 60% of the total worldwide consumption of the gas. But urea is usually made by reacting CO2 with ammonia — most of which is made with hydrogen derived from fossil fuels. Researchers such as Peter Styring, a chemical engineer at the University of Sheffield, UK, are working with industrial partners to develop catalysts and reaction conditions that will allow the production of urea directly from nitrogen, water and CO2. But currently, the urea industry is still a net source of CO2.
In the quest to make more materials from CO2 without adding to the greenhouse problem, chemists’ first challenge is to overcome the molecule’s stability. Unlike a carbon atom in isolation, which has four electrons that are available to form bonds, the carbon in CO2 has given up all four to the tight grip of the oxygen atoms. That grip has to be loosened before the carbon can form new bonds — and that takes energy. “If it energy comes from fossil fuels, it’s pointless,” says Styring. “Everything has to be from renewable energy.”
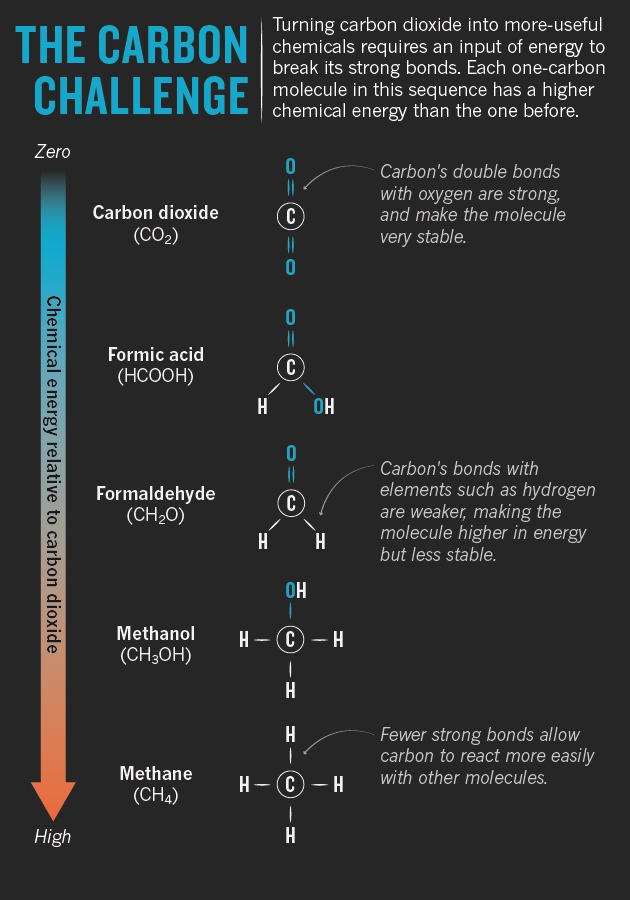
This energy cost of separating the carbon and oxygen atoms is so exorbitant that some researchers in the field simply sidestep the problem. They focus on products such as calcium carbonate (CaCO3), which can be made by combining CO2 with calcium compounds in low-energy reactions that leave the carbon–oxygen bonds relatively undisturbed. Calcium carbonate also happens to have a large potential market: it is used as a construction material and for whitening factory-produced paper. And because it is more inert than CO2, it could be used to store the gas over long time scales — something that already happens naturally in limestone deposits and coral reefs. “Nature has used mineralization to store millions of billions of tonnes of CO2,” says Martin Devenney, chief operating officer at Calera, a green-energy company in Los Gatos, California.
Most of the calcium carbonate now used in industry, an estimated 15 billion tonnes per year, comes from mining such deposits. But since 2013, Calera has been operating a demonstration facility that produces the material from CO2 and carbide residue: an industrial waste that comes from the production of polyvinyl chloride (PVC). Calera first adds water to the residue to extract calcium hydroxide, then bubbles CO2-rich flue gas from a nearby industrial plant through the solution to obtain pure calcium carbonate, which is turned into fibre cement boards that can be used in construction.
After factoring in energy costs, says Devenney, the company’s process captures about 170 kilograms of CO2 for every tonne of calcium carbonate manufactured — versus zero kilograms for every tonne that is mined.
In Asia, meanwhile, manufacturers licensing technology developed by Asahi Kasei Chemicals are using CO2 to produce about 660,000 tonnes of polycarbonates annually, roughly 14% of the global total. Polycarbonates are plastics used in products ranging from water bottles to spectacle lenses. They are usually made in a reaction involving phosgene (COCl2): a toxic compound that is infamous for its use as a poison gas in the First World War. It is typically produced by combining chlorine with carbon monoxide made by burning coal. The Asahi Kasei process replaces phosgene with CO2 — overcoming the molecule’s stability by putting it through three intermediate transformations.
Asahi Kasei’s process is still unable to make polycarbonate chains that are long enough for applications such as water bottles. But it is cost-competitive with the standard method, and according to Aresta’s estimates, reduces indirect CO2 emissions from 6 tonnes for every tonne of polycarbonate produced to 1 tonne.
Yet another promising area for low-energy CO2 utilization is in the production of polyols: a group of sugar-like molecules that are often used as the raw material for polymers such as polyurethanes, which are then used in mattresses, adhesives, coatings, refrigerator insulation and Spandex. Polyols are mostly made from precursor molecules known as epoxides. But Novomer, a green-chemistry company in Waltham, Massachusetts, has developed a cobalt-based catalyst that allows half of the epoxides to be replaced with CO2. “Our catalyst will not work without the carbon dioxide,” says Peter Shepard, executive vice-president of Novomer. The Novomer process is currently being tested at a plant in Houston, he says, where it is producing 3,000–4,000 tonnes of polyols per year while generating about one-third of the CO2 that would be produced through conventional methods. And the company has begun designing a facility with a capacity of 100,000 tonnes per year, scheduled for completion in 2017.
An uphill task
Despite these successes, the demand for low-energy products such as polycarbonates and inorganic carbonates is relatively limited. The big money is in hydrocarbons, which consist of one or more carbon atoms, often arranged in long chains or complex rings, surrounded by hydrogen atoms. As well as their many other uses, hydrocarbons are ubiquitous in petrol, diesel, jet fuel and just about every other liquid energy source on Earth — a market roughly 14 times larger than that for non-fuel chemicals.
Making hydrocarbons out of CO2 is a “completely different story” from making low-energy products, says Aresta. In addition to the energy required to break the carbon atom free from oxygen so that it can link up with other carbons, the process needs a source of cheap hydrogen — which currently comes from fossil fuels. And it is not easy to control the reactions to get a target hydrocarbon molecule instead of a related molecule that is one carbon shorter or longer. “This is tricky,” says Song.
Still, researchers are making progress. Since 2010, for example, Song has developed a series of catalysts based on iron and cobalt that can bring CO2 molecules close together with hydrogen molecules at 300 °C and about 10 atmospheres, forming ethylene, propylene and butane2. Because his experiments are still being done in the laboratory, Song cannot yet say how much his process could reduce greenhouse-gas emissions when carried out on an industrial scale. But any headway could have a large effect: these three chemicals are among the most heavily used in the chemical industry, says Song, with demand measured in the hundreds of millions of tonnes per year.
Song is also developing palladium–copper catalysts that can efficiently turn CO2 and hydrogen into methanol. “Mother Nature makes biomass and it takes months and years,” he says. “We can do it in seconds.”
As with other efforts to make fuels from CO2, however, the challenge is to do it efficiently: even in the laboratory, the known methods consume far more energy than the resulting fuels can provide. Among the most vexing problems is how to obtain the hydrogen. Right now, the choices are to extract the gas from fossil fuels, a source that is cheap but hardly green, or to make it by splitting water, a process that is both energy intensive and costly.
“We need at least 15 years of good research” to do better, estimates Aresta. And even then, he says, the conversion will probably not have a net benefit for climate unless the manufacturing plants can make extensive use of cheap energy from solar, wind and other renewables. “If we don’t use these kinds of energy, we will never be able to go to large-scale deployment,” he says.
Fuels and future
Some companies are already trying to do just that. In Reykjavik, for example, Carbon Recycling International (CRI) makes methanol from CO2 and hydrogen using the emissions and electricity from a neighbouring geothermal power plant. The CO2 in this case comes not from fossil fuel but from carbonate rocks baking in Earth’s heat deep underground. The hydrogen comes from the electrolysis of water — a source that would be prohibitively expensive if the power plant’s electricity were not so abundant. CRI currently produces about 5 million litres of methanol per year, or about 1.5% of the global production of methanol, which has 95% less CO2 emissions than petrol. The advantage for Iceland is that methanol, being a liquid fuel, is a much easier way to export the country’s wealth of geothermal energy than, say, laying an underground electricity cable to Europe. But for CRI, the plant is a test bed for wider deployment of its CO2-to-fuels technology, which it plans to market in other countries such as Germany as a way to capture and store large amounts of renewable energy at times of low demand. “It’s a matter of accessing energy at the right time,” says K.-C. Tran, cofounder and chief executive of CRI.
In most places, because renewable energy isn’t widely and cheaply available, the fuels from CO2 are still not competitive with those currently being made from crude oil, says Jim Yang Lee, a chemical engineer at the National University of Singapore. “It solves certain, localized problems, assuming you have certain resources,” he says, “but it’s not solving the global CO2 problem.”
The response from proponents is that it does not have to solve the problem all on its own: if some researchers manage to make progress with CO2-to-fuels conversion, they argue, while CO2-based chemical manufacturing continues to expand, CO2 utilization could make a noticeable dent in greenhouse-gas emissions in the near future.
This year, Styring evaluated various scenarios of CO2 utilization3. If 100% of urea, 30% of minerals, 20% of specific chemicals and polymers, 10% of methane and 5% of diesel and aviation fuels were supplied by currently known CO2-utilization methods, he estimates that around 1.34 gigatonnes of CO2 would be consumed per year. This equals around 83% of the IPPC’s 2030 global target for emissions reductions through CO2 capture and storage. “These are very conservative estimates,” says Styring. “It is likely that the impact will be much greater.”
For this to happen, researchers acknowledge that it will take far more than clever chemistry. Governments and industries worldwide might have to consider CO2 utilization along with storage, or even implement a carbon tax to help synthetic fuels stay competitive. “If you have to pay for CO2 to go into the atmosphere, then the story will change,” says Song.
Aresta is optimistic. “If we are able, in 20 years from now, to use solar and wind energy in a concrete way,” he says, “and use it to bring back CO2 into fuels, we can very, very easily reduce the emissions of CO2 by greater than 10%.”
With gigatonnes of the gas pouring into the atmosphere each year, and with the consequences for global climate becoming increasingly obvious, the Carbon XPRIZE would reward technologies that can convert CO2 emissions from coal and natural-gas power plants into useful products such as alternative building materials, fuels and raw material for the manufacture of plastics and other chemicals.
The invitation should have plenty of takers: a growing number of companies and research chemists are already pursuing that goal. In Reykjavik, what looks like an overgrown playground climbing frame is actually a small chemical plant that turns CO2 into methanol: a fuel that can also be used to manufacture products ranging from paints to wrinkle-resistant textiles. In Houston, Texas, another small plant is turning CO2 into materials that go on to become coatings and adhesives. And in Tokyo, Japan, Asahi Kasei Chemicals is widely licensing its technique for turning CO2 into the polycarbonate plastics used in bulletproof glass, spectacle lenses and electronic parts.
Using this greenhouse gas as a raw material is an idea that many scientists once dismissed as hopeless, says Chunshan Song, a chemical engineer at Pennsylvania State University, University Park. As a practical matter, he says, “lots of people believed that nothing could be done with CO2 utilization” after the stuff went up the smokestack.
As a source of carbon, sceptics argued, the gas was far more difficult and expensive to obtain than the petroleum, coal and natural gas that now provide the raw material for most chemical manufacturing. And even if CO2 could be captured cheaply enough, converting such a stable molecule into more-useful chemicals would generally require lots of energy, which might well come from fossil-fuel plants. The conversion could cost a fortune and make more CO2 than it consumed.
But the balance is starting to shift. New conversion technologies are allowing the energy-hungry chemical reactions to proceed more efficiently. Renewable sources such as solar and wind can increasingly supply that energy at a competitive cost without the carbon penalty. And solutions to the capture problem may be forthcoming if governments follow the recommendations of the Intergovernmental Panel on Climate Change (IPCC) and mandate carbon capture and storage — grabbing the CO2 coming out of power plants and other industries, and locking it away underground. But rather than simply burying it, companies could defray the cost of capture by putting the gas to productive use.
Michele Aresta, a chemist at the University of Bari in Italy, estimates that if currently known processes were deployed most efficiently and at the greatest possible scale, they could directly use some 300 million tonnes of CO2 per year, while indirectly reducing emissions by around a gigatonne per year — roughly 5% of the total net emissions1. This is hardly a total solution to the CO2 problem, he says, but it is substantial nonetheless. And that is not counting the additional benefits, which include a shift away from relying on fossil fuels as a source of carbon for materials and chemicals, as well as the use of CO2-derived fuels to store and transport energy from wind, solar and other intermittent sources.
The Carbon XPRIZE may well accelerate these developments. But research into CO2 conversion has already been receiving support from funding agencies worldwide. Since 2009, for example, the German Ministry of Education and Research has pumped in around €100 million (US$110 million) to support research on the use of CO2 in chemical and synthetic-fuel production. In 2011, the US Department of Energy invested $106 million in various CO2-utilization projects. The European Union has lined up a 2020 Horizon Prize worth €1.5 million for a technology that demonstrates viable CO2 reuse. And over the next five years, China is expected to invest some 30 billion yuan ($4.7 billion) in CO2 recycling in the coal, steel, cement and paper industries.
Low-hanging fruit
Industrial uses of CO2 have been around for generations. Every year, about 20 million tonnes of the gas are used ‘as is’: for example, it’s the ‘fizz’ in fizzy drinks, and it can also be used as a high-pressure solvent to clean electronics. But gas used in this way quickly returns to the atmosphere, where it contributes to global warming.
Another 114 million tonnes of CO2 go into the production of urea fertilizer every year, accounting for more than 60% of the total worldwide consumption of the gas. But urea is usually made by reacting CO2 with ammonia — most of which is made with hydrogen derived from fossil fuels. Researchers such as Peter Styring, a chemical engineer at the University of Sheffield, UK, are working with industrial partners to develop catalysts and reaction conditions that will allow the production of urea directly from nitrogen, water and CO2. But currently, the urea industry is still a net source of CO2.
In the quest to make more materials from CO2 without adding to the greenhouse problem, chemists’ first challenge is to overcome the molecule’s stability. Unlike a carbon atom in isolation, which has four electrons that are available to form bonds, the carbon in CO2 has given up all four to the tight grip of the oxygen atoms. That grip has to be loosened before the carbon can form new bonds — and that takes energy. “If it energy comes from fossil fuels, it’s pointless,” says Styring. “Everything has to be from renewable energy.”
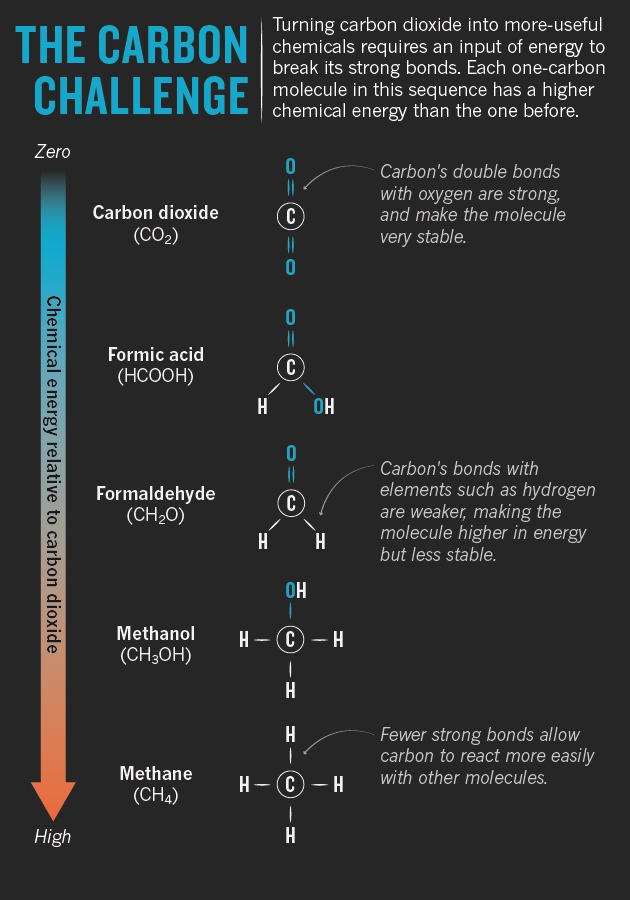
This energy cost of separating the carbon and oxygen atoms is so exorbitant that some researchers in the field simply sidestep the problem. They focus on products such as calcium carbonate (CaCO3), which can be made by combining CO2 with calcium compounds in low-energy reactions that leave the carbon–oxygen bonds relatively undisturbed. Calcium carbonate also happens to have a large potential market: it is used as a construction material and for whitening factory-produced paper. And because it is more inert than CO2, it could be used to store the gas over long time scales — something that already happens naturally in limestone deposits and coral reefs. “Nature has used mineralization to store millions of billions of tonnes of CO2,” says Martin Devenney, chief operating officer at Calera, a green-energy company in Los Gatos, California.
Most of the calcium carbonate now used in industry, an estimated 15 billion tonnes per year, comes from mining such deposits. But since 2013, Calera has been operating a demonstration facility that produces the material from CO2 and carbide residue: an industrial waste that comes from the production of polyvinyl chloride (PVC). Calera first adds water to the residue to extract calcium hydroxide, then bubbles CO2-rich flue gas from a nearby industrial plant through the solution to obtain pure calcium carbonate, which is turned into fibre cement boards that can be used in construction.
After factoring in energy costs, says Devenney, the company’s process captures about 170 kilograms of CO2 for every tonne of calcium carbonate manufactured — versus zero kilograms for every tonne that is mined.
In Asia, meanwhile, manufacturers licensing technology developed by Asahi Kasei Chemicals are using CO2 to produce about 660,000 tonnes of polycarbonates annually, roughly 14% of the global total. Polycarbonates are plastics used in products ranging from water bottles to spectacle lenses. They are usually made in a reaction involving phosgene (COCl2): a toxic compound that is infamous for its use as a poison gas in the First World War. It is typically produced by combining chlorine with carbon monoxide made by burning coal. The Asahi Kasei process replaces phosgene with CO2 — overcoming the molecule’s stability by putting it through three intermediate transformations.
Asahi Kasei’s process is still unable to make polycarbonate chains that are long enough for applications such as water bottles. But it is cost-competitive with the standard method, and according to Aresta’s estimates, reduces indirect CO2 emissions from 6 tonnes for every tonne of polycarbonate produced to 1 tonne.
Yet another promising area for low-energy CO2 utilization is in the production of polyols: a group of sugar-like molecules that are often used as the raw material for polymers such as polyurethanes, which are then used in mattresses, adhesives, coatings, refrigerator insulation and Spandex. Polyols are mostly made from precursor molecules known as epoxides. But Novomer, a green-chemistry company in Waltham, Massachusetts, has developed a cobalt-based catalyst that allows half of the epoxides to be replaced with CO2. “Our catalyst will not work without the carbon dioxide,” says Peter Shepard, executive vice-president of Novomer. The Novomer process is currently being tested at a plant in Houston, he says, where it is producing 3,000–4,000 tonnes of polyols per year while generating about one-third of the CO2 that would be produced through conventional methods. And the company has begun designing a facility with a capacity of 100,000 tonnes per year, scheduled for completion in 2017.
An uphill task
Despite these successes, the demand for low-energy products such as polycarbonates and inorganic carbonates is relatively limited. The big money is in hydrocarbons, which consist of one or more carbon atoms, often arranged in long chains or complex rings, surrounded by hydrogen atoms. As well as their many other uses, hydrocarbons are ubiquitous in petrol, diesel, jet fuel and just about every other liquid energy source on Earth — a market roughly 14 times larger than that for non-fuel chemicals.
Making hydrocarbons out of CO2 is a “completely different story” from making low-energy products, says Aresta. In addition to the energy required to break the carbon atom free from oxygen so that it can link up with other carbons, the process needs a source of cheap hydrogen — which currently comes from fossil fuels. And it is not easy to control the reactions to get a target hydrocarbon molecule instead of a related molecule that is one carbon shorter or longer. “This is tricky,” says Song.
Still, researchers are making progress. Since 2010, for example, Song has developed a series of catalysts based on iron and cobalt that can bring CO2 molecules close together with hydrogen molecules at 300 °C and about 10 atmospheres, forming ethylene, propylene and butane2. Because his experiments are still being done in the laboratory, Song cannot yet say how much his process could reduce greenhouse-gas emissions when carried out on an industrial scale. But any headway could have a large effect: these three chemicals are among the most heavily used in the chemical industry, says Song, with demand measured in the hundreds of millions of tonnes per year.
Song is also developing palladium–copper catalysts that can efficiently turn CO2 and hydrogen into methanol. “Mother Nature makes biomass and it takes months and years,” he says. “We can do it in seconds.”
As with other efforts to make fuels from CO2, however, the challenge is to do it efficiently: even in the laboratory, the known methods consume far more energy than the resulting fuels can provide. Among the most vexing problems is how to obtain the hydrogen. Right now, the choices are to extract the gas from fossil fuels, a source that is cheap but hardly green, or to make it by splitting water, a process that is both energy intensive and costly.
“We need at least 15 years of good research” to do better, estimates Aresta. And even then, he says, the conversion will probably not have a net benefit for climate unless the manufacturing plants can make extensive use of cheap energy from solar, wind and other renewables. “If we don’t use these kinds of energy, we will never be able to go to large-scale deployment,” he says.
Fuels and future
Some companies are already trying to do just that. In Reykjavik, for example, Carbon Recycling International (CRI) makes methanol from CO2 and hydrogen using the emissions and electricity from a neighbouring geothermal power plant. The CO2 in this case comes not from fossil fuel but from carbonate rocks baking in Earth’s heat deep underground. The hydrogen comes from the electrolysis of water — a source that would be prohibitively expensive if the power plant’s electricity were not so abundant. CRI currently produces about 5 million litres of methanol per year, or about 1.5% of the global production of methanol, which has 95% less CO2 emissions than petrol. The advantage for Iceland is that methanol, being a liquid fuel, is a much easier way to export the country’s wealth of geothermal energy than, say, laying an underground electricity cable to Europe. But for CRI, the plant is a test bed for wider deployment of its CO2-to-fuels technology, which it plans to market in other countries such as Germany as a way to capture and store large amounts of renewable energy at times of low demand. “It’s a matter of accessing energy at the right time,” says K.-C. Tran, cofounder and chief executive of CRI.
In most places, because renewable energy isn’t widely and cheaply available, the fuels from CO2 are still not competitive with those currently being made from crude oil, says Jim Yang Lee, a chemical engineer at the National University of Singapore. “It solves certain, localized problems, assuming you have certain resources,” he says, “but it’s not solving the global CO2 problem.”
The response from proponents is that it does not have to solve the problem all on its own: if some researchers manage to make progress with CO2-to-fuels conversion, they argue, while CO2-based chemical manufacturing continues to expand, CO2 utilization could make a noticeable dent in greenhouse-gas emissions in the near future.
This year, Styring evaluated various scenarios of CO2 utilization3. If 100% of urea, 30% of minerals, 20% of specific chemicals and polymers, 10% of methane and 5% of diesel and aviation fuels were supplied by currently known CO2-utilization methods, he estimates that around 1.34 gigatonnes of CO2 would be consumed per year. This equals around 83% of the IPPC’s 2030 global target for emissions reductions through CO2 capture and storage. “These are very conservative estimates,” says Styring. “It is likely that the impact will be much greater.”
For this to happen, researchers acknowledge that it will take far more than clever chemistry. Governments and industries worldwide might have to consider CO2 utilization along with storage, or even implement a carbon tax to help synthetic fuels stay competitive. “If you have to pay for CO2 to go into the atmosphere, then the story will change,” says Song.
Aresta is optimistic. “If we are able, in 20 years from now, to use solar and wind energy in a concrete way,” he says, “and use it to bring back CO2 into fuels, we can very, very easily reduce the emissions of CO2 by greater than 10%.”
You can return to the main Market News page, or press the Back button on your browser.